History of Ferroelectric Doped HfO2 and ZrO2
In 2006, Tim Böscke was investigating doped HfO2 and ZrO2 dielectrics for DRAM capacitor applications at Qimonda (formerly Infineon), a manufacturer of dynamic random-access memories (DRAMs). Specifically, he tried to replicate results just published by Akira Toriumi’s group, which reported a maximum dielectric constant for 10 nm thick HfO2 films containing 4-5 atomic percent Si. Toriumi had suggested that the higher dielectric constant could be caused by a tetragonal phase (t phase, space group: P42/nmc), which was difficult to distinguish from a non-centrosymmetric orthorhombic phase (oIII phase, space group: Pca21). At that time, in the phase diagrams of HfO2 and ZrO2 at room temperature and atmospheric pressure, only centrosymmetric nonpolar phases were reported, such as the t-phase or the monoclinic phase (m-phase, space group: P21/c). Böscke found a voltage-dependent capacitance enhancement for a particular concentration when measuring the capacitance as a function of voltage for HfO2-based capacitors with different Si concentrations, which could not be explained by normal paraelectric behavior. Through a detailed electrical and structural characterization of the capacitor, the enhancement was found to be due to ferroelectric switching. A previously unreported non-centrosymmetric orthorhombic Pca21 phase was suggested to cause this behavior. Subcycle and saturation polarization, field cycling endurance, fatigue, imprint, and piezoresponse experiments confirmed the ferroelectric properties.
For the first three years, the focus was on integrating ferroelectric HfO2 into non-volatile memory devices, such as a ferroelectric field-effect transistor (FeFET) or a one-transistor/one-capacitor ferroelectric random access memory (FeRAM), because the initial results were obtained at a memory device manufacturer. Consequently, the first FeFET transistor was produced in 2007 using the 65 nm technology node, which was significantly smaller than any reported FeFET at the time. Unfortunately, Qimonda’s bankruptcy delayed further research; however, NaMLab and Fraunhofer IPMS-CNT continued the work, and GlobalFoundries in Dresden, Germany began fabricating FeFET devices at an even smaller 28 nm technology node.
In 2011, five years after the initial experiments, the first publication reporting these exciting findings appeared, followed by several others. The observation of similar ferroelectric performance in HfxZr1-xO accelerated progress in the field, as this material offers a wide range of properties from mostly dielectric (x ≈ 1) to ferroelectric (x ≈ 0.5) to antiferroelectric (x ≈ 0). The research community had to be convinced that a simple oxide with a binary or ternary fluoride structure could have ferroelectric properties. Many groups joined the research effort to identify the proposed Pca21 oIII phase by diffraction in transmission electron microscopy, optimize the ferroelectric properties, and understand ferroelectricity’s origin in HfO2. Over the past decade, the applications of ferroelectric materials have expanded from ferroelectric capacitors, transistors, and tunneling interconnects for non-volatile memory applications to negative capacitors, logic-in-memory, neuromorphic computing, supercapacitors, and applications based on pyroelectrics or piezoelectrics. A rapidly growing number of publications is evidence of the strong research activity in this area.
Ongoing research
During the last two years, the main focus of developing ferroelectric HfO2-based materials is the detailed understanding of the ferroelectric properties in thin doped HfO2 layers. A variety of dopant materials were studied in addition to a mixed Hf1-xZrxO2. Deposition techniques included atomic layer deposition and physical vapor deposition. The ferroelectric orthorhombic Pca21 phase of HfO2 is formed when the material is crystallized with a certain dopant or oxygen concentration at the phase boundary between the monoclinic and the tetragonal/cubic phase and is enhanced through mechanical confinement. Scanning transmission electron microscopy and electron diffraction methods confirmed the structure. Continuous research aims to understand the root cause of this previously unknown phase. Here, dopant and oxygen content, which are directly related to stress and strain in the layer, play an essential role in phase stabilization. A linear relation between strain and the coercive field of the phase transition field was found (Figure 1).
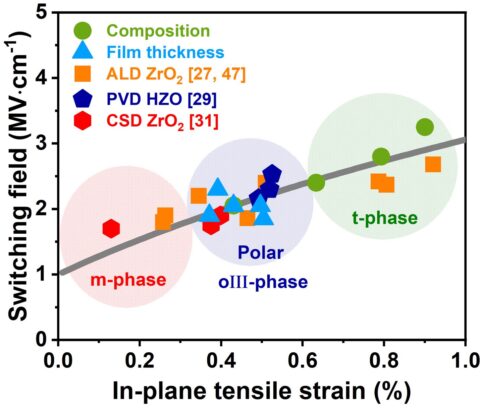
These parameters impact thermal stability and film reliability. Based on these results, significant improvements in the film performance could be achieved. Ab initio simulations by partners at the Munich UAS confirmed the influence of the factors mentioned above on the phase stability of ferroelectric HfO2 and proposed a similar relationship of barrier height for ferroelectric switching as a function of strain (Figure 2).
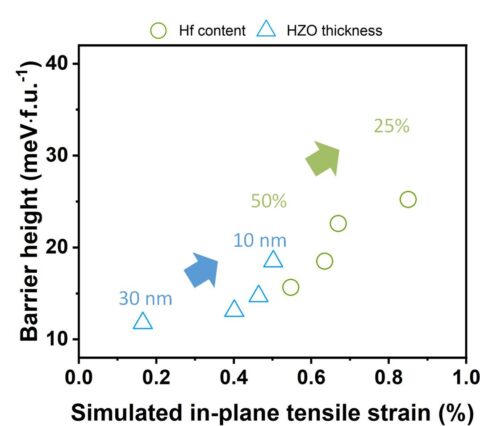
Depending on the dopant material, the polarization hysteresis showed a maximum remanent polarization value between 15-40 μC/cm². The highest values were obtained for lanthanum-doped HfO2 with TiN electrodes. Piezo-response force microscopy (Oak Ridge Nat. Laboratory/Univ. Nebraska), in conjunction with transmission electron microscopy measurements, revealed domains within single grains with a diameter of ~20-30 nm for 10 nm thick films. For ZrO2, a field-induced transition from non-polar to polar grains is found, as expected for a tetragonal to orthorhombic phase transition (Figure 3). The polycrystalline structure of the films caused a varying polarization orientation within the layer. The size distribution of the grains follows a Poisson distribution, resulting in a grain size-dependent coercive field and Curie temperature.
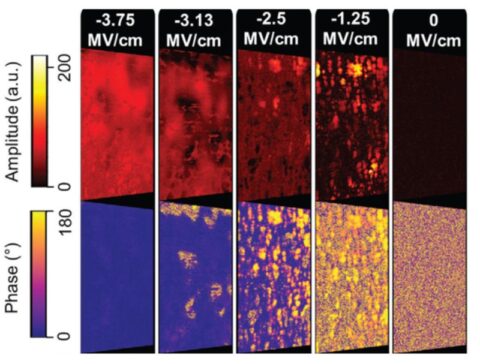
Future studies will focus on the structural basis of the ferroelectric properties, their impact on the ferroelectric switching behavior, and how device cycling performance can be improved.
Cooperation:
Fraunhofer IPMS-CNT, Dresden (Germany), RWTH Aachen (Germany), UAS Munich (Germany), GLOBALFOUNDRIES Dresden (Germany), IMEC (Belgium), Oak Ridge National Labs (USA), Dalin University (China), North Carolina State University Raleigh (USA), Tokyo Institute of Technology (Japan)